Abstract
The Tambora eruption (1815 AD) was one of the major eruptions of the last two millennia and has no equivalents over the last two centuries. Here, we collected an extensive network of early meteorological time series, climate simulation data and numerous, well-replicated proxy records from Eastern Canada to analyze the strength and the persistence of the Tambora impact on the regional climate and forest processes. Our results show that the Tambora impacts on the terrestrial biosphere were stronger than previously thought, and not only affected tree growth and carbon uptake for a longer period than registered in the regional climate, but also determined forest demography and structure. Increased tree mortality, four times higher than the background level, indicates that the Tambora climatic impact propagated to influence the structure of the North American taiga for several decades. We also show that the Tambora signal is more persistent in observed data (temperature, river ice dynamics, forest growth, tree mortality) than in simulated ones (climate and forest-growth simulations), indicating that our understanding of the mechanisms amplifying volcanic perturbations on climates and ecosystems is still limited, notably in the North American taiga.
Export citation and abstract BibTeX RIS

Original content from this work may be used under the terms of the Creative Commons Attribution 3.0 licence.
Any further distribution of this work must maintain attribution to the author(s) and the title of the work, journal citation and DOI.
Introduction
On 10 April 1815, the Tambora volcano, located in the Sumbawa island in Indonesia, entered into the culminating phase of a large Plinian eruption which had huge local and global impacts on climate, the environment and human societies (Harington 1992, Oppenheimer 2003, Raible et al 2016). Massive injection of at least 60 teragrams (Tg) of SO2 into the atmosphere produced stratospheric sulfate aerosols which decayed in concentration over three years and shielded the earth from incoming solar radiation (Self et al 2004). Consequently, global land temperatures dropped by about 1 °C in 1816, which is known as the 'year without a summer', and remained low for 6–10 years according to simulation studies (Raible et al 2016). This climatic perturbation occurred during a period of reduced solar irradiance (Dalton minimum) which was also impacted by other strong volcanic eruptions (Wagner and Zorita 2005), thus culminating in one of the coldest episodes of the 'Little Ice Age'.
Figure 1. Data location. The number of sites with specific tree-ring proxy records is within brackets. RW Liv. is ring width chronologies from living trees, RW Sub. is ring width chronologies from subfossil trees, MXD is ring maximum density chronologies, LME is Last Millennium Ensemble.
Download figure:
Standard image High-resolution imageAlthough the Tambora eruption is quite recent in historical terms, its impacts are much better constrained in Europe than elsewhere because of the denser network of historical sources, proxy records and early meteorological stations (Brugnara et al 2015). Furthermore, many discrepancies exist on the intensity and duration of the Tambora climatic impact as detected by different observed (e.g. weather observations, tree-ring proxies) and simulated records (Büntgen et al 2015). These discrepancies remain largely unexplained because of the poor mechanistic understanding of the responses of the climate and the biosphere at the regional level. For example, the Tambora impact on the carbon cycle is poorly understood. The atmospheric CO2 concentration was slightly reduced for 15 years after the Tambora erupted (MacFarling Meure et al 2006), likely because the carbon uptake over land increased. This was driven by the lower temperatures, which could have reduced ecosystem respiration in northern latitudes and increased net primary production in the tropics (Tjiputra and Otterå 2011, Kandlbauer et al 2013). However, there is no consensus on the terrestrial biosphere responses and associated mechanisms at regional level (Raible et al 2016). For example, we do not know how northern forest ecosystems reacted to the Tambora abrupt perturbation in terms of wood biomass assimilation and forest demography (i.e. tree mortality and recruitment), which are significant components of the regional carbon budget.
This paper focuses on the Tambora impacts in Eastern Canada (figure 1) where many individual records and sources show that the volcanic perturbation on climate was particularly strong. The Hudson's Bay Company and the Moravian missionary archives report extremely cold temperatures and thick and persistent sea-ice conditions in the Hudson Bay and along the Labrador coast from October 1815 to March 1818, at the beginning of a generally cold 60 year period (Wilson 1992, D'Arrigo et al 2003). These harsh climatic conditions contributed to poor hunting success, crop failure and famines afflicting native people, as well as European traders and colonists from Ontario to the Labrador coast (Suffling and Fritz 1992, D'Arrigo et al 2003). In southern Quebec, severe winters between 1816 and 1819 caused the formation of exceptional ice bridges on the St. Lawrence River near Quebec City for four consecutive years (Houle et al 2007). The Tambora impact in Eastern Canada is also visible in biological proxy records. The lowest 1816 anomalies recorded in a Northern Hemisphere network of tree-ring density measurements come from the Quebec-Labrador Peninsula (Briffa et al 1998). Simultaneously and in the same region, we detect the beginning of a 40 year regime shift to the lowest values of the last millennium in ring width chronologies (Gennaretti et al 2014c). Unfavorable climatic conditions during the first half of the 19th century are also detectable in pollen datasets (Viau et al 2012), δ18O cellulose of Sphagnum mosses (Bilali et al 2013), and reconstructions of spruce height growth (Vallée and Payette 2004). These climatic, historical and biological impacts are in line with the idea, corroborated by model simulation experiments, that Eastern Canada may be especially sensitive to volcanic forcing, if an amplification by subsequent North Atlantic sea-ice-ocean feedbacks takes place (Otterå et al 2010, Miller et al 2012). However, a comprehensive study merging several kinds of observed and simulated annually-resolved data from Eastern Canada during the Tambora time has yet to be carried out.
Here, we present an extensive network composed of early meteorological observations, climate simulations, and river and tree-ring proxy records to analyze the Tambora impacts in Eastern Canada. We evaluate the propagation of the of the Tambora signal through the regional climate and the forest ecosystems. As it has been shown for other regions or at the global scale (Segschneider et al 2013, Büntgen et al 2015), we hypothesize that the persistence of volcanic signals in biological records and in ecosystem carbon uptake may be longer than in the climate system. We also reconstruct tree mortality in the Eastern Canadian taiga during the early 19th century to analyze whether the Tambora eruption severely affected forest demography and structure with long-term consequences. We then discuss the mechanisms that could have amplified the volcanic impact and that are not taken into account by ecophysiological models. Finally, we compare the likely impacts on forest carbon uptake of Tambora-like eruptions simulated under past (early 19th century) and current (last decades) climate conditions.
Materials and methods
Hydroclimate observations
We collected observed data from early meteorological records and annals of river break-up dates. The meteorological records come from historical and modern observations from several observers and three locations in Southern Quebec: Montreal, Quebec City and Fort Coulonge (figure 1). These observations were compiled by Slonosky (2014, 2015), www.ncdc.noaa.gov/paleo-search/study/16336 into two unique time series for the St. Lawrence Valley of daily minimum and maximum temperature values. Here we used the mean of the two time series over the 1803–2010 period (St. Lawrence Tmean hereafter). The river break-up dataset consists of the first break-up dates of river estuaries on the west coast of the Hudson and James Bays in the 18th and 19th centuries. These data were compiled by Moodie and Catchpole (1975) and used in a regional multi-proxy climate reconstruction (Guiot 1985). We retained three rivers and locations with more complete data over the 1736–1866 period: Churchill Factory, Fort Albany and Moose Factory (figure 1, dataset S1 available at stacks.iop.org/ERL/13/034017/mmedia).
Simulated climate
Thirteen climate simulations with all transient forcings of the Last Millennium Ensemble project (LME; Otto-Bliesner et al 2016, www.cesm.ucar.edu/projects/community-projects/LME/) were used to further evaluate the temporal and spatial climate impacts of the Tambora eruption over Eastern Canada. The LME offers the possibility to analyze a large ensemble of simulations varying for initial conditions (background state of the climate system at the beginning of the model runs) but conducted with the same up-to-date model version (CESM-CAM5_CN developed primarily at the National Center for Atmospheric Research) and using the same set of forcings over the 850–2005 period. In this way, we limit uncertainties due to the use of different models and forcing records which would complicate our analysis, but at the same time we can explore the natural forcing and internal variability of the climate system with multiple simulations. For each simulation, we extracted the following climate variables: reference height (two meters) mean-maximum-minimum temperature, total precipitation and net solar flux at surface. These data were used to produce annual time series plots, monthly anomalies plots, and maps. A simple post-processing method was applied to the data of the grid node in the middle of our study region in Eastern Canada (figure 1). This consisted in correcting each variable by the difference between its 1950–2005 average and the average value in the NRCan observational dataset (the gridded interpolated Canadian database of daily minimum–maximum temperature and precipitation for 1950–2015; Hutchinson et al 2009, http://cfs.nrcan.gc.ca/projects/3/4).
Tree-ring proxies and forest carbon
An extensive network of tree-ring temperature-sensitive proxy records covering the central Quebec-Labrador peninsula and always including the 1800–1850 period was assembled to analyze the Tambora impact in Eastern Canada (figure 1). This network includes data from living and subfossil spruce trees. All records from living trees go back at least to 1800 AD and come from Nicault et al (2014a, 2014b) and Naulier et al (2015), namely, 56 ring width chronologies (hereafter RW Liv.) calculated using the age-band standardization method (Briffa et al 2001), 19 maximum density chronologies (MXD) and three δ18O and δ13C chronologies (rings were sampled at a two-year resolution for the isotopic chronologies). Additionally, ring width data were derived from subfossil black spruces (tree remains into lakes) collected from six sites (Gennaretti et al 2014c). These data were assembled into six site-specific and two cambial age-specific chronologies covering the 910–2011 period (RW Sub.) using the regional curve standardization pivot correction method which was designed to reduce the impact of varying sampling heights in the used material (Autin et al 2015). Decadal tree-mortality rates at these same six sites were reconstructed from the outermost tree-ring dates of the subfossil trees. However, it must be noted that the outermost tree-rings are eroded with the time (Gennaretti et al 2014b) and that, as a consequence, the retrieved dates of tree deaths are antedated by some years.
Figure 2. Hydroclimate observations. St. Lawrence annual Tmean time series (a) and 1816 monthly anomalies ((b); black: 1816 anomalies, grey: 90% confidence intervals computed with data over the 1803–1814 and 1818–2010 period). River estuaries' first break-up date time series ((c); thin lines: annual values, bold lines: three-year running means; note that the y-axis is inverted and lower values correspond to later first break-up in spring) and their 1816 anomalies ((d); pdfs are empirical probability density functions and are computed with the running mean data over the 1736–1814 and 1818–1866 period, horizontal lines are 1816 values). In (a) and (c) vertical lines are important eruption years (see table 1; Tambora is in red). DOY is the day of year.
Download figure:
Standard image High-resolution imageTable 1. Years of large eruptions plotted in the figures and derived from Gao et al (2008, global total stratospheric sulfate aerosol injection >15 Tg), Crowley and Unterman (2013, satellite aerosol optical depth >0.1), Esper et al (2013, eruptions classified as large events) or Sigl et al (2013, 2015, eruptions classified as large events).
Year | Volcano | Location |
---|---|---|
1739 | Shikotsu | Japan |
1755 | Katla | Iceland |
1783 | Laki | Iceland |
1809 | Unknown | Unknown |
1815 | Tambora | Indonesia |
1831 | Babuyan | Philippines |
1835 | Cosiguina | Nicaragua |
1854 | Shiveluch | Russia |
1875 | Askja | Iceland |
1883 | Krakatau | Indonesia |
1902 | Santa Maria | Guatamala |
1907 | Ksudach | Russia |
1912 | Katmai | USA |
1913 | Colima | Mexico |
1956 | Bezymianny | Russia |
1963 | Agung | Indonesia |
1991 | Pinatubo | Indonesia |
Figure 3. LME simulated values for the grid node in the middle of our study region in Eastern Canada. Mean 2 m temperature annual time series ((a); black: mean of the 13 members, grey: inter-member spread) and 1816 monthly anomalies in the mean ensemble time series relative to the monthly-specific median value ((b); black: 1816 anomalies, grey: 90% confidence intervals computed with data over the 1790–1814 and 1818–2005 period of the mean ensemble time series). Mean total daily precipitation time series (c) and 1816 monthly anomalies (d). In (a) and (c) vertical lines are important eruption years (see table 1; Tambora is in red).
Download figure:
Standard image High-resolution imageFigure 4. Average 1816 global anomaly fields of the 13 member LME ensemble relative to the 25 year period between 1790 and 1814. We show anomalies of mean 2 m temperature ((a); °C), mean total daily precipitation ((c); mm day−1), and mean net solar flux at surface ((e); W m−2). White areas denote non-significant anomalies (one-sample Wilcoxon test with p > 0.05; n = 13). In (b), (d) and (f) the value of the grid point in the middle of our study region in Eastern Canada is indicated by a red horizontal line and is compared to the distribution of the values in the maps (PDFs).
Download figure:
Standard image High-resolution imageFigure 5. Tree-ring proxy time series in Eastern Canada over the 1800–1850 time period. In (a)–(d) we see single site (19 MXD chronologies in (a); 3 δ18O chronologies in (b); 56 RW Liv. and 6 RW Sub. chronologies in (c); 3 δ13C chronologies in (d)) and mean multisite chronologies. All data are transformed in z-scores. The red shadow in (a) and (c) highlights the years impacted by the Tambora. Two cambial age-specific multisite chronologies are also shown in (c) for the subfossil material (bold green dotted lines; cambial ages < 50 years and cambial ages > = 50 years). The independent multisite chronologies are compared in (e). The (f) plot shows the 1816 anomalies (crosses) relative to the distribution of the data over the 1800–1814 and 1818–1850 period (quartiles and extreme values). In (a)–(e) vertical lines are important eruption years (see table 1; Tambora is in red). MXD is ring maximum density chronologies, RW Liv. and Sub. are ring width chronologies from living and subfossil trees, respectively.
Download figure:
Standard image High-resolution imageThe impact of the Tambora eruption on boreal forest aboveground carbon assimilation in eastern Canada was firstly evaluated through the computation of a wood biomass production index (MWI; g yr−1, Boucher et al 2017) at the 19 sites where both tree-ring width and density measurements were available:
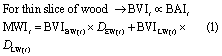
where BVI is the basal volume increment (cm3), BAI is the basal area increment (cm2), D is the wood density (g cm−3), and EW and LW are the earlywood and the latewood fractions, respectively. Secondly, the MAIDEN ecophysiological forest model (Misson 2004, Gea-Izquierdo et al 2015), recently adapted for boreal black spruce sites (Gennaretti et al 2017), was used to simulate the forest gross primary production (GPP) in the study region in Eastern Canada during the Tambora time. We used as MAIDEN inputs daily minimum–maximum temperature and precipitation values extracted by the 13 LME simulations and CO2 concentration data obtained by interpolating to daily resolution the ice core CO2 record of MacFarling Meure et al (2006). The parameters used to run MAIDEN were sampled from their posterior distributions described in Gennaretti et al (2017).
Statistical significance of the 1816 anomaly
The significance of the 1816 anomaly just after the 1815 Tambora eruption was verified in all the used datasets by comparing the 1816 values to the distribution of the data excluding the years most impacted by the Tambora (1815–1817). For climate simulations, we also compared the 1816 anomalies in the study area against the distribution of the 1816 anomalies from elsewhere (see figures 2–5).
Results
The large amount of collected data, including hydroclimate observations, climate simulations and tree-ring proxies, is well-spread over Eastern Canada (figure 1). The St. Lawrence annual mean temperature (Tmean) time series indicates that the Tambora eruption induced a significant cooling over three years in Southern Quebec (1816–1818) with maximum 1816 negative anomalies up to −5 °C in spring and summer (figure 2). At the same time, the first break-up date of the main rivers on the west coast of the Hudson/James Bays was delayed by about 10 d and was especially late in 1817 indicating cold winter/spring conditions (figure 2). Comparatively, climate simulations of the LME project highlight that the Tambora produced negative anomalies in Eastern Canada on both temperature and precipitation time series (figure 3), but these anomalies lasted only one year and the 1816 temperature anomalies were more negative in winter than in summer (the opposite was shown by the St. Lawrence Tmean). The LME simulations also highlight that Eastern Canada was one of the regions of the world displaying the coldest temperature anomalies in 1816 which was a cold year almost everywhere (figures 4(a)–(b)). These anomalies in Eastern Canada were not associated with a reduction of solar irradiance (figures 4(e)–(f)), suggesting that they were caused by some climate feedbacks or teleconnections affecting the atmospheric circulation. The 1816 global picture is much more complicated for precipitation anomalies (figures 4(c)–(d)). Some regions of the world displayed wet anomalies and others displayed dry anomalies, such as Eastern Canada.
Tree-ring proxy records in Eastern Canada were strongly impacted by the Tambora eruption and, to a lesser extent, by the other large eruptions of the first half of the 19th century (figure 5). The strength and the persistence of the impact depend on the proxy. The MXD time series show the lowest indexes in 1816 and 1817, which are followed by fast recovery. Conversely, the RW time series drop down in 1816 and remain low for about 12 years, with the exception of normal growth in 1822. The recovery process was almost completed in 1828 but the 1831 Babuyan and the 1835 Cosiguina eruptions reduced the growth again. A similar response is shown by the isotopic proxies (δ18O and δ13C), with particularly low values in 1824 and 1825 (these years are negative anomalies for all proxies). Although RW data come from a large region in Eastern Canada (figure 1), the behavior of the RW time series (figure 5(c)) is extremely consistent whatever the site, the material used (living or subfossil trees), and the tree cambial age (subfossill trees with cambial ages < 50 years or cambial ages > = 50 years).
The RW data from subfossil trees allow the analysis of the Tambora impact on tree mortality. These data are extremely robust due to the high sample depth (overall replication always over 200 trees; figure 6(a)), which declines up to the Tambora eruption suggesting that a massive tree mortality was likely linked to this event. Figure 6(b) indicates that 19% of the trees died in the decade ending in 1815 and 15% in the decade ending in 1805. Note that tree mortality is slightly antedated here because it is reconstructed with the outermost tree-ring dates and subfossil trees lose, through erosion, an average of 3.2 outermost rings per century, a rate subjected to high variations according to the conditions of preservation (Gennaretti et al 2014b). This indicates that the actual death date of the trees should be closer to 1816 and that the mortality caused by the Tambora (post 1815) should be likely higher than 20%, meaning four times higher than the background level. The subsequent eruptions (e.g. the 1835 Cosiguina eruption) were not associated to any detectable mortality events likely because a large portion of the most vulnerable trees had already died after the Tambora eruption.
If we use the computed wood biomass production index at 19 sites as a proxy of the boreal forest aboveground carbon assimilation in eastern Canada, we can conclude that carbon dynamics are very similar to ring width variations (figure 7). This is because the coefficient of variation (ratio of the standard deviation to the mean) of the basal volume increments is nine times larger than that of wood density values (see equation 1). Consequently, the Tambora impact seems to last 12 years such as for RWs. This long-term impact is not captured by the MAIDEN simulations of black spruce GPP values during the Tambora time (figure 8). Indeed, these simulations only show one important negative anomaly in 1816 with a median percent reduction of GPP values of −11.6%. This lack of persistence is likely due to a combination of reasons: (1) the LME time series used as MAIDEN input underestimate the persistence of the Tambora impact on the regional climate (compare figures 2(a) and 3(a)); (2) the real mechanism forcing the physiological persistence of poor growth over several years two centuries ago remains unidentified and is not taken into account by ecophysiological forest models.
Figure 6. Sample depth ((a); number of trees) and tree mortality rate ((b); percentage of tree mortality over the total sample depth per each ten year class) in the subfossil material (all six sites). The Tambora year is the red line.
Download figure:
Standard image High-resolution imageFigure 7. Wood biomass production index (MWI; values transformed in z-scores) over the 1800–1850 period at the 19 sites where both tree-ring width and density measurements are available. Vertical lines are important eruption years (see table 1; Tambora is in red).
Download figure:
Standard image High-resolution imageFigure 8. Black spruce forest GPP time series simulated by MAIDEN in the middle of our study region in Eastern Canada (a). The average of 130 simulations is shown (black line; ten simulations per each LME-member climate record sampling the MAIDEN sensitive parameters from their plausible distributions for the study region; see Gennaretti et al 2017) as well as the 80% confidence interval (dark grey shadow) and the outlier simulations (light grey thin lines). Vertical lines are important eruption years (see table 1, Tambora is in red). The boxes and whiskers in (b) show the distribution of the simulated values over the 1800–1814 and 1818–1850 period compared to the distribution for the year 1816. The GPP percent reduction in 1816 relative to the other years is shown in (c). In (b) and (c), the distributions obtained in the experiment 1 (fixed CO2 concentration equal to the 1985–2005 mean) and the experiment 2 (CO2 as in experiment 1 and temperature and precipitation time series modified by constant deltas: +0.56 °C and +0.74% respectively) are also shown.
Download figure:
Standard image High-resolution imageDiscussion
Our results illustrate that the legacy effects of the Tambora eruption in Eastern Canada depend on the record. There are significant differences between hydroclimate observations and the specific proxy records. The amplitude of the 1816 anomaly in the early temperature observations is similar to that in the ring width and δ13C time series. In contrast, ring density records appear to be overly sensitive (figure 9), a result consistent with the findings of Tingley et al (2014) pointing out the impact on wood density of reduced light availability after volcanic eruptions. However, ring width and δ13C values show a much longer persistence of the Tambora impact (12 years) than temperature observations (three years) and ring density (two years). Ring width records are known to have a smoothed delayed response to volcanic events (Esper et al 2015) because carbon dynamics in trees are generally the results of processes occurring during the current and the previous years (e.g. tree damage and recovery processes, accumulation of stored non-structural carbohydrates; see Carbone et al 2013). On the other hand, δ13C values are a proxy of the plant intrinsic water use efficiency (Moreno-Gutiérrez et al 2012, Farquhar et al 1989, McCarroll and Loader 2004), which is directly related to the net photosynthetic rate and inversely related to the stomatal conductance. Consequently, the low δ13C values after the Tambora eruption indicates a slow photosynthetic rate that lasted for some years and/or an increase of stomatal conductance after foliage losses (see Berninger et al 2000). These findings highlight that different biases may be present in reconstructions based on specific proxies and that multi-proxy approaches should be preferred. The post-Tambora three-year persistence detected in annual temperature observations may seem quite short because simulations studies have shown that Eastern Canada could be sensitive to strong volcanic eruptions, especially in volcanic active periods such as the early 19th century. Indeed, eruptions may be linked to the inception of longer-term feedbacks (e.g. variation in the Atlantic thermohaline circulation) determining the Atlantic multidecadal variability (Otterå et al 2010, Miller et al 2012, Schleussner and Feulner 2013). However, if we exclude tree-ring widths and isotopes, no other annually resolved observation (MXD, historical sources, river and sea-ice observations) shows a Tambora impact longer than four years in Eastern Canada (see data collected for this study and references cited in the introduction). Consequently, our results suggest a longer persistence of the Tambora signal in some specific Eastern Canada tree-ring proxy records than in the regional climate, which is in accordance with what has been found in Europe (Büntgen et al 2015).
Tree-ring data are excellent climate proxy records and, more specifically, are archives of changes in forest processes. Here, using our ring width and density data, we suggest that the carbon uptake of the black spruce trees in Eastern Canada was dampened for 12 years following the Tambora (figure 7). Such a long impact on forest processes is also testified by the δ13C time series (figure 5). Simulation studies have already shown that volcanic perturbations are longer on land carbon pools than on global climate because of slower recovery processes (Segschneider et al 2013). However, our findings highlight that the Tambora legacy in Eastern Canada may be even longer than one or two decades because the eruption also triggered a massive tree mortality episode (figure 6). Consequently, the Tambora impact on tree density could have persisted until the present because, in the study region, forest grows slowly and was almost unable to regenerate by seed during the Little Ice Age (Payette et al 2008). Indeed, the complete maturation of black spruce embryos needs at least 800 growing degree-days (above 5 °C; Meunier et al 2007), a threshold still not attained today in the center of the study region. Thus, each mortality event may be associated with a persistent shift in forest structure and composition (Gennaretti et al 2014a).
Figure 9. 1816 anomalies (crosses) in specific records: St. Lawrence Tmean and summer (July–August means) temperature values, Last Millennium Ensemble (LME) 2 m mean temperature, ring maximum density chronologies (MXD), ring width chronologies from living trees (RW Liv.), ring width chronologies from subfossil trees (RW Sub.), the mean first break-up date at Fort Albany and Moose Factory, δ18O and δ13C. All records are scaled to have same mean and variance than St. Lawrence Tmean over the 1800–1850 period. Boxes and whiskers show quartiles and extreme values of the data over the 1800–1814 and 1818–1850 period. For the river break-up record, the cross highlights the 1817 anomaly which is the lowest anomaly following the Tambora in this record. In the right side of the figure, the St. Lawrence Tmean record was preprocessed to have a two-year resolution and to be comparable to the δ18O and δ13C tree-ring records.
Download figure:
Standard image High-resolution imageWe suggest two complementary hypotheses to explain the mortality event and the long persistence of the Tambora impact on the forest even if more favorable climatic conditions were reestablished four-five years after the eruption. The first hypothesis is related to the occurrence of frost damages, followed by long-term recovery processes to rebuild the canopy photosynthetic capacity. This mechanism would be in part similar to that suggested by Fayle et al (1992). Immature shoot development during short growing seasons combined with needle and bud erosion during the cold winters in 1816-17 most likely damaged upper crowns of dominant trees, leading to growth form regression and increased stem mortality. The plausibility of this mechanism is supported by the finding of Arseneault and Payette (1997) that growth forms and ring widths of black spruce at a treeline site were severely impacted for several decades following a series of strong volcanic eruptions during the 1450s (Esper et al 2017). Similarly, an extended episode of tree mortality in a Labrador treeline forest stand during the early 19th century has been attributed to progressive erosion of foliage and buds by severe winter conditions (Payette 2007). The second hypothesis is related to a likely frost disturbance of the 'rootable' soil layer. The 19th century is known to be a period with intense ice-wedge and permafrost activity in Northeastern Canada (Kasper and Allard 2001). Although permafrost is generally absent from forested sites in Quebec-Labrador, due to high winter precipitation and snow retention (Payette 2001), short-term cooling events (three-four years), such as the one produced by the Tambora, could have resulted in deeper freezing and shorter frost-free season. This mechanism could have been amplified by a thin snow cover in 1816, as suggested by climate simulations (figure 3(d)), and by the presence of an insulating moss-Sphagnum mat, as it is the case in several taiga forest stands, that would have preserved the frozen soil.
Our results illustrate that the Tambora imprint in Eastern Canada is more persistent on observed data than on simulated ones (climate and forest-growth simulations). The LME mean temperature records show a stronger 1816 anomaly than climate observations (figure 9), but the low anomalies last one year only (figure 3). Indeed, at the regional level, decadal climate responses to volcanic eruptions may be controlled by the internal climate variability and by the background state of the climate system (e.g. the climatic impact of an eruption on a specific region can differ according to the preceding atmospheric and oceanic state; Zanchettin et al 2013). Consequently, the clear 6–10 year post-Tambora negative temperature anomalies simulated by different GCMs at the global scale (Raible et al 2016) are much noisier at the regional scale. The absence of a reduction of the solar flux at surface over Eastern Canada in 1816 (figure 4(e)) seems to represent a LME bias because reduced light availability is suggested by our observed data (ring density and δ13C low values). The short-term temperature anomaly in the LME records contributed to the short-term reduction of black spruce primary production simulated by MAIDEN in the study area (figure 8). However, this short-term effect may be primarily explained by the fact that both hypotheses formulated in the previous paragraph are linked to mechanisms that are not taken into account by process-based vegetation models. Consequently, although MAIDEN is able to consider the influence of carbon stored during previous years on stand carbon dynamics (Gennaretti et al 2017), our GPP simulations only show a post-Tambora anomaly of one year. The 11.6% GPP reduction simulated by MAIDEN in 1816 may had partially or completely been counteracted by a concomitant reduction in soil respiration (not simulated by MAIDEN), which is known to occur in northern latitudes following volcanic eruptions (Tjiputra and Otterå 2011, Kandlbauer et al 2013). For all these reasons, the response of the studied boreal ecosystem in terms of net ecosystem production following the Tambora and the investigation of the mechanisms amplifying the volcanic perturbation remain interesting topics for future studies.
Ultimately, our study may help anticipate the effects of a future Tambora-like eruption on the eastern Canadian boreal forest carbon uptake. At the global scale, some simulation studies have shown that Tambora-like eruptions occurring in the 21th century could delay the upcoming warming by approximately two decades and could significantly increase the terrestrial global carbon uptake (Tjiputra and Otterå 2011, Schurer et al 2015). This global picture is much more uncertain at the regional scale. For our study region, we compared the 1816 GPP anomalies simulated by MAIDEN for black spruce forests (figure 8) with those simulated with modified versions of the early 19th century input climate. In the first experiment, we fixed the atmospheric CO2 concentration data to the mean value of the 1985–2005 period (358 ppm; +74 ppm relative to 1800–1850; MacFarling Meure et al 2006). The simulated GPP values increased by about 50 g C m−2 year−1 (figure 8(b)) but the 1816 relative percent reduction (figure 8(c)) remained practically unchanged. In the second experiment, in addition to the CO2 modification, we also modified the LME temperature and precipitation time series to have their 1800–1850 mean equal to their 1985–2005 mean: +0.56 °C for temperature and +0.74% for precipitation. Although these are small climatic deltas, the average GPP values increased an additional 25 g C m−2 year−1 (figure 8(b)). However, the 1816 relative percent reduction remained very similar and only just slightly smaller (the median values shifted from −11.6% to −11.0%; figure 8(c)). These analyses suggest that immediately after Tambora-like events, similar or slightly reduced impacts to those of the early 19th century can be expected on the Eastern Canada forest carbon uptake. However, the persistence of the impact and the tree mortality should be reduced in the current and future warmer climate if the triggering mechanisms are those suggested above. Furthermore, the regenerative potential of black spruce forests following disturbances is higher today than in the 19th century (Meunier et al 2007).
Conclusion
In this study, we collected a large amount of data from different and complementary sources over Eastern Canada, to provide an in-depth evaluation of the Tambora impact on climate and forest processes. We confirm the results of previous studies for other regions, showing that the terrestrial biosphere is much more sensitive to volcanic eruptions than the climate system. We point out that the Tambora legacy on the structure of the North American taiga could have persisted until the present. Indeed, the eruption caused a mortality event of the dominant trees during an unfavorable period for forest regeneration. We suggest two mechanisms to explain the observed tree mortality and the persistence of the Tambora impact on the forest carbon uptake: (1) frost damages eroding the spruce growth forms; (2) deeper freezing and shorter frost-free season in the soil. These mechanisms are not considered by process-based vegetation models, which underestimate the Tambora legacy on northern ecosystems.
Acknowledgments
This project has received funding from the European Union's Horizon 2020 research and innovation programme under the Marie Sklodowska-Curie grant agreement No 656896.