ABSTRACT
Cosmogenic isotope records indicate that a solar-cycle modulation persists through extended periods of very low sunspot activity. One immediate implication is that the photospheric field during such grand minima did not consist entirely of ephemeral regions, which produce a negligible amount of open magnetic flux, but continued to have a large-scale component originating from active regions. Present-day solar and heliospheric observations show that the solar wind mass flux and proton density at the coronal base scale almost linearly with the footpoint field strength, whereas the wind speed at Earth is uncorrelated with the latter. Thus a factor of ∼4–7 reduction in the total open flux, as deduced from reconstructions of the interplanetary magnetic field (IMF) during the Maunder Minimum, would lead to a similar decrease in the solar wind densities, while leaving the wind speeds largely unchanged. We also demonstrate that a decrease in the strengths of the largest active regions during grand minima will reduce the amplitude of the Sun's equatorial dipole relative to the axial component, causing the IMF strength to peak near sunspot minimum rather than near sunspot maximum, a result that is consistent with the phase shift observed in the 10Be record during the Maunder Minimum. Finally, we discuss the origin of the 5 yr periodicity sometimes present in the cosmogenic isotope data during low and medium amplitude cycles.
Export citation and abstract BibTeX RIS
1. INTRODUCTION
Cosmogenic isotopes, produced by galactic cosmic rays impinging on the Earth's atmosphere, provide a proxy for long-term solar activity. The cosmic-ray flux is largely determined by the strength of the interplanetary magnetic field (IMF); since weaker fields are less effective in impeding the diffusion of charged particles through the heliosphere, the flux is generally greater during times of low solar activity, as is the formation rate of cosmogenic isotopes. During the past 9300 yr, there have been as many as ∼26 periods of enhanced 10Be and 14C production lasting ∼50–100 yr, implying corresponding episodes of reduced sunspot activity (McCracken et al. 2011). The best known of these "grand minima" is the 1645–1715 Maunder Minimum, when sunspots almost disappeared and auroral sightings became rare (Eddy 1976; Suess 1979; Kopecký & Kuklin 1987; Ribes & Nesme-Ribes 1993; Hoyt & Schatten 1996; Cliver et al. 1998; Letfus 2000; Kovaltsov et al. 2004). Significantly, annual-resolution measurements of the 10Be concentration in the Dye 3 and NGRIP (Greenland) ice cores indicate that 11 and 22 yr periodicities persisted throughout the Maunder Minimum as well as the 1420–1540 Spörer Minimum (Beer et al. 1998; McCracken et al. 2004, 2011; Berggren et al. 2009). However, as noted by Beer et al. (1998) and Usoskin et al. (2001), the dips in the 10Be concentration during the Maunder Minimum did not coincide with the times when the occasional sunspots were seen, but were roughly in antiphase with this residual activity.
Steinhilber et al. (2010) have used the time-dependent cosmic-ray modulation potential ϕmod derived from the 10Be record to reconstruct the long-term variation of the IMF intensity. Here, ϕmod∝vw/k determines the energy loss and thus the spectrum of cosmic rays in the force-field approximation (Gleeson & Axford 1968), where inward diffusion through the IMF irregularities with coefficient k is balanced by outward convection by the solar wind with speed vw. Taking k∝B−1.8IMF based on comparisons between neutron monitor data and in situ measurements of the total IMF strength BIMF (see also Caballero-Lopez et al. 2004; McCracken 2007; Rouillard & Lockwood 2007), Steinhilber et al. (2010) concluded that the Sun's open flux or radial IMF component at Earth, BE, has increased by a factor of ∼4.5 since 1700, from ∼0.7 nT to ∼3.2 nT (see also McCracken 2007). A similar increase was found by Scherer & Fichtner (2004) using a one-dimensional, multifluid model that takes into account the motion of the heliospheric interface (which, however, turns out to have negligible effect on the cosmic-ray modulation, unlike the IMF strength).
Given the extremely low level of sunspot activity at that time, these estimates for the IMF strength during the Maunder Minimum (which depend on the form of the cosmic-ray diffusion coefficient and, to a lesser extent, on the assumed solar wind speed) may seem surprisingly high. Indeed, if the Sun's open magnetic flux is taken to scale as the sunspot number or if it is arbitrarily parameterized in terms of long transfer timescales from rapidly decaying active-region fields (see Solanki et al. 2000, 2002; Krivova et al. 2007; Vieira & Solanki 2010), the IMF would vanish through most of the Maunder Minimum. The difficulty becomes much less severe if it is recognized that the axial dipole moments of the active regions of a given cycle are cumulative and survive even after all activity has ceased, in the form of the polar fields and the axisymmetric component of the Sun's open flux. Taking the rate of active region emergence to be 30 times lower than in solar cycle 21 and employing flux transport simulations, Wang & Sheeley (2003a) obtained values of BE averaging about 0.5 nT during the Maunder Minimum, only slightly smaller than those deduced by Steinhilber et al. (2010).
It has sometimes been suggested that ephemeral regions—the very small, short-lived, randomly oriented bipoles scattered everywhere over the photosphere—may have been the source of the IMF during the Maunder Minimum (Solanki et al. 2002; Cliver & Ling 2011). However, as noted in Wang & Sheeley (1991) and Wang et al. (2005) and as demonstrated again in Section 2 of this paper, the dipole moments of ephemeral regions are far too small to contribute significantly to the IMF, unless (contrary to observations) they are all aligned in the same direction.
The phase shift exhibited by the 10Be time series during the Maunder Minimum, indicating that the IMF reached its lowest intensity near the maximum rather than the minimum of the 11 yr activity cycle, provides a potentially important clue about the sources of the Sun's large-scale field at that time. During recent cycles, the IMF strength near sunspot maximum has been determined by the Sun's equatorial dipole and quadrupole components, whose sources are large active regions. The question arises whether a decrease in the number or strength of such active regions can change the shape of the IMF variation during very low amplitude cycles.
Owens & Lockwood (2012) and Owens et al. (2012) have presented a model for the solar cycle variation of the IMF, in which the source term for the open flux is taken to be proportional to the sunspot number, while its loss rate varies as the tilt angle of the heliospheric current sheet (HCS), which approaches 90° near sunspot maximum. Taking the evolution of the tilt angle to be the same during every cycle, they find that the loss term becomes relatively dominant in weak cycles, leading to a minimum in the open flux near sunspot maximum. This model assumes that a residual source of open flux, attributed to coronal mass ejections (CMEs), is present even when the sunspot number is zero.
McCracken et al. (2002) have noted the presence of a 5 yr periodicity in the 10Be data during several low amplitude cycles, occurring at the end of the Maunder Minimum, during the 1800–1830 Dalton Minimum, and in the early part of the twentieth century. At these times, the IMF apparently underwent a broad dip near the maximum as well as near the minimum of the sunspot cycle. Although not commented on by McCracken et al. (2002), as many as one-half of the cycles showing the 5 yr periodicity (viz. cycles −2, 8, 15, and 17) in fact had peak yearly sunspot numbers Rmax > 100, so that the periodicity does not seem to be exclusively a property of "low" sunspot activity. Usoskin et al. (2006) have suggested that the additional enhancements in the 10Be concentration were caused by large, ground-level solar proton events, whose dates and fluences have been inferred by McCracken et al. (2001) from nitrate anomalies in polar ice. As is evident from Figure 3 of Usokin et al., however, the timing of the proton events generally does not match that of the peaks in the 10Be record seen near sunspot maximum.
The purpose of this study is to clarify some of the questions raised above about the nature of the IMF and solar wind, and in particular their relationship to the photospheric field, during periods of very low sunspot activity. We begin by showing that ephemeral regions are unlikely to contribute significantly to the Sun's open flux or even to provide a realistic "floor" to the IMF variation (Section 2). We then use present-day observations to infer that the solar wind mass fluxes and densities were much lower during grand minima than in recent cycles, but that the wind speeds remained more or less unchanged (Section 3). In Section 4, we employ flux transport calculations to explore the relationship between the solar cycle evolution of the open flux and the number and strength of its active region sources, in an attempt to understand the phase shift in the 10Be time series during the Maunder Minimum. Section 5 focuses on the origin of the 5 yr periodicity detected in the cosmogenic isotope record. Our results are summarized in Section 6.
2. EPHEMERAL REGIONS AND THE OPEN MAGNETIC FLUX
The Sun's open flux is determined by the lowest-order multipoles of the photospheric field, and above all by its dipole (l = 1) component. As we now demonstrate, the net dipole moment of the multitude of ephemeral regions on the Sun is much smaller than that associated with active regions and their remnants (which include the polar fields).
The properties of ephemeral regions have been extensively studied by Harvey-Angle (1993) using daily magnetograms from the National Solar Observatory at Kitt Peak, and by Hagenaar (2001) and Hagenaar et al. (2003) using Michelson Doppler Imager full-disk magnetograms recorded every 96 minutes. According to these studies, ephemeral regions have average pole separations of ∼9 Mm and lifetimes of a few hours; the distribution function of their fluxes follows an exponential with an average of 1.1 × 1019 Mx. The total flux emerging over the entire photosphere in the form of ephemeral regions was estimated as 2–6× 1022 Mx day−1 by Harvey-Angle (1993) and as 5 × 1023 Mx day−1 by Hagenaar (2001). Most importantly for determining their contribution to the open flux, ephemeral regions with fluxes below a few times 1019 Mx have no preferred axial orientation (Hagenaar et al. 2003). It should be remarked that Harvey (1994) found that the axial dipole moments of her sample of ephemeral regions showed a slight tendency to have the opposite sign to those of larger active regions with the normal Hale–Joy polarity orientations; unlike Solanki and his coworkers (Solanki et al. 2002; Krivova et al. 2007; Vieira & Solanki 2010), we interpret this result to mean that any preferred axial orientation lies below the noise level of the measurements.
On the assumption that ephemeral regions have random axial orientations, we now derive an upper limit to their contribution to the Sun's net dipole strength. Because the equatorial (or nonaxisymmetric) component of a dipole field, being subject to the photospheric differential rotation, undergoes a much faster diffusive decay than the axial (or axisymmetric) component, we need to consider only the latter. The axial dipole strength of a single bipole having total flux Φ0 and linear pole separation Δs is given by dax = (3/8π)Φ0(Δs)sin γcos L0/R3☉, where γ is the angle between the bipole axis and the east–west line and L0 is the latitude at which the bipole is centered (Wang & Sheeley 1991). Taking γ = 45° and L0 = 30° as typical values for these parameters, we have

Let the total rate of flux emergence in the form of ephemeral regions be denoted by dΦ/dt. The mean square value of Dax(t), the net axial dipole strength of all of the ephemeral regions on the Sun, grows at a rate

The axial dipole decays on the timescale τd ≃ R2☉/(2κ) ≃ 15 yr, where κ ∼ 500 km2 s−1 is the turbulent diffusion rate associated with the nonstationary supergranular convection (see Leighton 1964; Wang & Sheeley 1991; Wang et al. 2002). The axial dipole component of the ensemble of ephemeral regions thus saturates when it attains the root mean square amplitude
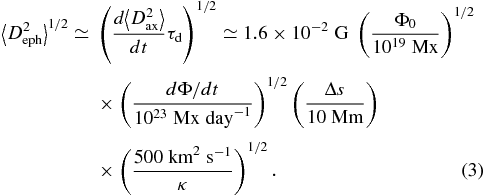
For comparison, the contribution of large active regions and the polar fields to the Sun's axial dipole strength has been as much as 4 G during recent cycles (Wang et al. 2002; see also Figure 2 below), which is two orders of magnitude greater than 〈D2eph〉1/2, for any reasonable choice of the emergence rate dΦ/dt of ephemeral region flux. It is therefore clear that ephemeral regions cannot be a significant contributor to the open flux unless the IMF strength falls by a factor of ∼100 below its contemporary values; in contrast, the decreases inferred from cosmogenic isotope records during grand minima are at most a factor of 10 (McCracken 2007). Again, this result is based on the observational finding that ephemeral regions have random tilt angles; if their north–south dipole moments all pointed in the same direction, Deph would be a factor of [(1/Φ0)(dΦ/dt)τd]1/2 ∼ 7500 larger, and ephemeral regions would be the dominant source of an extremely strong IMF.
That ephemeral regions were not the source of the IMF during the Maunder Minimum is also consistent with the persistence of an 11 yr modulation in the 10Be concentration. Unlike active regions, ephemeral regions emerge at a nearly constant rate through the solar cycle (Hagenaar et al. 2008; Schrijver et al. 2011), so that the modulation of the open flux must be due to the emergence and decay of active regions.
If ephemeral regions are regarded as providing a theoretical lower limit on the Sun's open flux, the resulting "floor" values of the radial IMF strength at Earth are much lower than those proposed by Svalgaard & Cliver (2007), Owens et al. (2008), and Cliver & Ling (2011), which range from ∼3 nT down to ∼0.6 nT. The actual floor, however, will be determined by the surviving polar fields at sunspot minimum and their associated axial dipole strength.
3. EFFECT OF A REDUCED PHOTOSPHERIC FIELD STRENGTH ON THE SOLAR WIND
A number of inferences about the nature of the solar wind during grand minima can be made simply by examining the behavior of the present-day wind as a function of the underlying photospheric field strength. As in Wang (2010), we now use mass and energy conservation along a flux tube and field line tracing to relate measurements of the proton bulk speed v, mass density ρ, and radial IMF strength B at Earth (subscript "E") to the corresponding parameters at the coronal base (subscript "0").
The mass flux density at the coronal base may be expressed in terms of that observed at Earth by

Similarly, the energy flux density at the coronal base is given by

where we have separated out the contribution of the gravitational potential energy from our definition of Fw and used the fact that the energy flux at Earth is dominated by the bulk kinetic energy.
To determine vE, ρE, and BE, we employ 8 hr averages of hourly measurements recorded between 1998 February and 2012 February by the Advanced Composition Explorer (ACE). We have not attempted to remove the contribution of CMEs; because of the large number of data points used, their effect on the overall statistics is expected to be small.
The footpoint field strengths are derived by extrapolating magnetograph data in the form of 27.3 day synoptic maps from the Mount Wilson Observatory (MWO) and the Wilcox Solar Observatory (WSO); as in Wang et al. (2009), we average together the two data sets. Let r denote heliocentric radius, L heliographic latitude, and ϕ Carrington longitude. The line-of-sight measurements are corrected for the saturation of the Fe i 525.0 nm line profile by multiplying them by the latitude-dependent factor (4.5 − 2.5sin 2L), and are deprojected by dividing by cos L (see Wang & Sheeley 1995; Ulrich et al. 2002, 2009). A potential-field source–surface extrapolation is then applied to map the field out to r = Rss = 2.5 R☉. Field lines that cross the source surface are considered to be "open"; their footpoint areas have been shown to coincide approximately with observed coronal holes throughout the solar cycle (see, e.g., Wang et al. 1996; Neugebauer et al. 1998; Liewer et al. 2004). Following the prescription of Schatten (1971), sheet currents are introduced into the region r > Rss by matching a potential field with l = 0 monopole component to |B(Rss, L, ϕ)| and then restoring the polarity of the field lines. The footpoint (R☉, L0, ϕ0) of the open field line that intersects the spacecraft is found by tracing inward from 1 AU, allowing for the solar rotation and resulting longitude shift during the wind propagation time (∝v−1E).
Figure 1(a) shows a scatter plot of log10(Fw0) against log10(B0). The solar wind energy flux at the coronal base is seen to increase almost linearly with the footpoint field strength, over a range of more than two orders of magnitude in both quantities (the dashed line indicates a slope of one in the log–log plot). Note that we have made no assumptions about the nature of the energy flux at the Sun in obtaining this empirical result, which therefore provides support for the hypothesis that the magnetic field is the main source of the heating and acceleration of the solar wind, even when the underlying photospheric field is very weak. For the present epoch, the median values of the footpoint field strength and energy flux density at the coronal base are 〈B0〉 = 11.3 G and 〈Fw0〉 = 6.1 × 105 erg cm−2 s−1, respectively. For an arbitrary footpoint field strength B0, the dashed-line fit in Figure 1(a) gives for the corresponding solar wind energy flux density

Figure 1. Scatter plots relating parameters of the contemporary solar wind (derived from ACE measurements) to the photospheric field strength. (a) Energy flux density at the coronal base, log10(Fw0) (105 erg cm−2 s−1), vs. footpoint field strength, log10(B0) (G). (b) log10(Fw0) vs. proton flux density at the coronal base, log10(n0v0) (1013 cm−2 s−1). (c) log10(n0v0) vs. log10(B0). (d) Wind speed at Earth, vE (km s−1), vs. log10(B0) (G). In (a)–(c), the dashed line corresponds to a slope of unity in the log–log plot. A dot is plotted for every 5° of longitude during Carrington rotations 1933–2119 (1998 February to 2012 February). An average of MWO and WSO measurements was employed for the photospheric field.
Download figure:
Standard image High-resolution imageFigure 1(b) shows a nearly perfect correlation between log10(Fw0) and log10(n0v0), where n0 denotes the proton density at the coronal base. This result, which follows directly from Equation (5), expresses the fact that a major portion of the energy flux at the coronal base goes into lifting the mass out of the Sun's gravitational well.
Figure 2. (a) Variation of |Dax| (G) and Deq (G) during 1977–2008 (cycles 21–23), derived by averaging MWO and WSO photospheric field measurements. The two dipole components have roughly comparable amplitudes, but are in antiphase with each other over the solar cycle. On yearly timescales, however, increases in Deq occurring after (before) polar field reversal are accompanied by increases (decreases) in |Dax|; thus the variation of Dax reinforces the tendency for the IMF strength to peak just after activity maximum, which is due mainly to the contribution of Deq. (b) Variation of the radial IMF strength at Earth, BE (nT), where the observations are from the OMNI 2 data set. The highest peaks in BE occur just after sunspot maximum and coincide approximately with those in Deq, plotted as dotted lines. All curves in this figure represent 82 day (three Carrington rotation) running means.
Download figure:
Standard image High-resolution imageAs confirmed by Figure 1(c), the statistical relationships in Figures 1(a) and (b) together imply that the mass flux density at the coronal base increases almost linearly with the footpoint field strength. The median value of n0v0 during 1998–2012 is 1.2 × 1014 cm−2 s−1. For an arbitrary footpoint field strength B0, the corresponding value of the proton flux density is

The scatter plot in Figure 1(d) demonstrates that there is no systematic relationship between the wind speed at Earth and the footpoint field strength. Instead (see, e.g., Wang & Sheeley 1990), vE shows a significant inverse correlation with the rate of flux-tube expansion in the corona, described by the parameter fss = (R☉/Rss)2(B0/Bss), where Bss denotes the field strength at the source surface.
Similar results are obtained using Ulysses data taken over a wide range of latitudes (see Figures 3 and 4 in Wang 2010). In particular, comparing the 1993–1996 and 2006–2008 polar passes (when the spacecraft was above |L| = 40°), we find that the median value of the footpoint field strength 〈B0〉 decreased by 31% (from 10.6 to 7.3 G) between the two sunspot minima; correspondingly, 〈Fw0〉 decreased by 26% (from 5.2 to 3.8 × 105 erg cm−2 s−1) and 〈n0v0〉 decreased by 23% (from 6.5 to 5.0 × 1013 cm−2 s−1). In contrast, the median wind speed fell by only 3% (from 763 to 740 km s−1), as the polar holes shrank slightly and 〈fss〉 increased from 4.2 to 5.1.
The 10Be-based reconstructions of Steinhilber et al. (2010) and Scherer & Fichtner (2004) and the flux transport simulations of Wang & Sheeley (2003a) suggest that the radial IMF strength was a factor of ∼4–7 lower during the Maunder Minimum than in the present day; the footpoint field strengths in coronal holes would have decreased by a similar amount, from B0 ∼ 10 G to B0 ∼ 2 G. Based on the scatter plots of Figure 1, we then deduce that both the energy and the mass flux densities at the Sun were a factor of ∼4–7 lower during the Maunder Minimum.
The persistence of an 11 yr modulation in the 10Be record implies that polarity reversals continued throughout the Maunder Minimum. Correspondingly, the polar holes would have continued to wax and wane, and the heliosphere would again have been dominated by high-speed wind near sunspot minimum but by slow wind near sunspot maximum. Observations during the 2007–2009 activity minimum indicate that the weakening of the polar fields was accompanied by a small decrease in the polar hole areas and high-latitude wind speed, by a widening of the equatorial band of low-speed wind, and at the same time by an increased occurrence of high-speed streams in the ecliptic (see McComas et al. 2008; Gibson et al. 2009; Kirk et al. 2009; Luhmann et al. 2009; de Toma 2011; Manoharan 2012). The smaller polar holes and wider streamer belt, as compared with the previous sunspot minimum, were due both to the weakness of the polar fields and to the lingering presence of active region remnants at low latitudes; the two effects combined to close down some of the polar hole flux, to increase the number and size of low-latitude holes, and to maintain a warped HCS (compare Figures 10 and 11 in Wang et al. 2009). The low-latitude fields eventually decay away on a timescale of 1–2 yr, as they are transported to midlatitudes and annihilated by rotational shearing and diffusion. In the absence of any further sunspot activity, the polar hole boundaries would reach a steady-state equilibrium in which the poleward meridional flow balances the equatorward diffusion of the polar cap flux, with faster flows leading to more concentrated polar fields and smaller polar holes (Sheeley et al. 1989; Petrie & Patrikeeva 2009). If the flow speeds were lower during the Maunder Minimum than in the present day, as suggested by the flux transport simulations of Wang & Sheeley (2003a) and Karak (2010) (see also Section 4), the polar holes would have been larger, not smaller, and the polar wind somewhat faster, not slower. Nevertheless, in the absence of sunspot activity, the Earth itself would have remained immersed in the slow wind belt surrounding the HCS, even while the rest of the heliosphere was permeated by high-speed wind.
4. SOLAR CYCLE VARIATION OF THE IMF STRENGTH DURING GRAND MINIMA
We now consider the possible origin of the phase shift observed in the 10Be time series during the Maunder Minimum, when the 10Be concentration peaked near sunspot maximum instead of at sunspot minimum.
As noted in Section 2, the time variation of the radial IMF strength approximately follows that of the Sun's total dipole strength Dtot(t) = (D2ax + D2eq)1/2, whose two components are related to the photospheric flux distribution B(R☉, L, ϕ, t) by




where the integrals are taken over all solid angle Ω. The axial component Dax varies in a manner similar to the Sun's polar fields, reaching its peak strength near sunspot minimum and vanishing near sunspot maximum. In contrast, the equatorial component Deq varies in phase with sunspot activity, while undergoing large fluctuations. The equatorial dipole strength is a function of both the level of sunspot activity and its distribution in longitude, and attains its peak values when large active regions emerge with their east–west dipole moments reinforcing each other (Wang & Sheeley 2003b). Thus, although the rate of active region emergence is greatest at sunspot maximum, the net equatorial dipole moment tends to peak somewhat later, when activity becomes concentrated in a smaller number of very strong regions having a relatively nonuniform distribution in longitude.
During recent cycles, Dax(t) and Deq(t) had roughly comparable amplitudes, with the peak strengths of the Sun's equatorial dipole component (reached in 1982, 1991, and 2003) being ∼30% larger than the axial dipole strengths during the activity minima of 1976, 1986, 1996, and 2008 (see Figure 2). Correspondingly, the total dipole and IMF strengths attained their highest values when Deq(t) peaked approximately 2 yr after the maximum of each cycle.
Because Dax(t) and Deq(t) vary out of phase with each other, it is apparent that the shape of the IMF variation over a solar cycle will in general be determined by the relative amplitudes of the two components. Thus, if the amplitude or peak strength of the equatorial dipole were significantly smaller than that of the axial dipole, the total dipole and IMF would reach their greatest strength near sunspot minimum rather than near sunspot maximum.
A single active region may be idealized as a bipole whose axial and equatorial dipole strengths are related through dax/deq ∼ tan γ < 1, where γ is the tilt relative to the east–west line. For a given total magnetic flux Φtot distributed equally among N bipoles obeying the Hale–Joy laws, the net axial dipole strength will scale as Ndax (because the individual axial dipole moments point in the same direction, either all northward or all southward), whereas the net equatorial dipole strength will scale as N1/2deq (because the individual east–west dipole moments are randomly oriented if the bipoles emerge at random longitudes). This suggests that if the active regions of a given cycle are relatively weak but still numerous enough to reverse the polar fields, the amplitude of the Sun's equatorial dipole component (∝N1/2) may be reduced sufficiently compared to the axial dipole (∝N) to cause the IMF strength to peak near sunspot minimum.
To explore this effect, we simulate the middle cycles of the Maunder Minimum by employing a procedure similar to that in Wang & Sheeley (2003a), but adopting two different forms for the source term. The time evolution of the radially oriented photospheric field is derived by numerically integrating the equation

where ∇2⊥ denotes the L and ϕ components of the Laplacian, ω(L) = 13.38 − 2.30sin 2L − 1.62sin 4L deg day−1 is the synodic rotation rate of the photospheric plasma (from Snodgrass 1983), κ = 500 km2 s−1 is the supergranular diffusion coefficient, v(L) is the meridional flow speed, and S(L, ϕ, t) is the source term representing flux emergence in the form of bipoles. The flow, of amplitude vm, is assumed to be directed poleward in each hemisphere and to vary with latitude as

so that the speed peaks at L = ±133 (see Wang et al. 2009). As discussed in Wang & Sheeley (2003a; see also Karak 2010), polarity reversals can be maintained throughout the Maunder Minimum by substantially reducing the flow speeds compared to present-day values, thereby maximizing the diffusive annihilation of leading-polarity flux at the equator and allowing more of the trailing-polarity flux to reach the poles. As in Wang & Sheeley (2003a), we set vm = 7.5 m s−1, roughly a factor of two smaller than measured during recent cycles (see Ulrich 2010, and references therein).
For the initial photospheric field, we arbitrarily adopt

corresponding to an axial dipole strength Dax(t = 0) = 0.6 G. The emerging flux is represented by idealized doublets having pole separations of 15°; their polarity orientations obey the standard Hale–Joy laws, with the tilt angles increasing with latitude according to sin γ = 0.6sin |L| (see Wang & Sheeley 1991). Within each cycle, the deposition rate is modulated so that it peaks ∼4 yr after the start of the cycle. The bipoles are distributed randomly over a 10° wide band centered at latitude L0 = ±10°; a random number generator is also used to assign a longitude and hemisphere to each bipole.
We consider two different scenarios for the relationship between the strength of the individual bipoles and their total number. First, let us suppose that 25 bipoles emerge during each cycle, with each bipole having a total flux Φ0 = 4 × 1022 Mx. For this "strong bipole" case, Figure 3 shows the time variation of the bipole deposition rate, total photospheric flux, axial and equatorial dipole components, and radial IMF strength at Earth over four 11 yr cycles. The total photospheric flux is here expressed as an average of |B(R☉, L, ϕ, t)| over the solar surface, while

where rE = 1 AU and we have used the fact that the open flux ends up distributed uniformly in latitude and longitude (Balogh et al. 1995; Smith & Balogh 2008).
Figure 3. Evolution of the solar magnetic field for the "strong bipole" case, where 25 bipoles each having flux Φ0 = 4 × 1022 Mx are deposited per 11 yr cycle. (a) Time variation of the total photospheric flux (solid curve), expressed as a surface-averaged field strength (G). Dotted curve indicates the number of bipoles deposited during each year of the cycle. (b) Evolution of the axial and equatorial dipole components of the photospheric field, Dax (solid curve) and Deq (dotted curve), where both quantities are in gauss. (c) Time variation of the radial IMF strength at 1 AU, BE (nT) (solid curve); dotted curve represents the equatorial dipole strength Deq (G).
Download figure:
Standard image High-resolution imageFrom Figure 3(b), we see that the amplitude of modulation of the equatorial dipole is comparable to or somewhat greater than that of the axial dipole, with Deq(t) exhibiting multiple peaks through the middle years of each cycle. Corresponding oscillations appear in the radial IMF strength (Figure 3(c)), with the highest values lying well above the solar minimum levels of BE(t) and the lowest values (or valleys between the peaks) lying below or at this level.
In our second experiment, we increase the number of bipoles deposited per cycle from 25 to 100, but decrease their strengths from Φ0 = 4 × 1022 Mx to Φ0 = 1 × 1022 Mx, so that the total flux emerging during each cycle is the same as in the previous simulation. As shown in Figure 4, the solar cycle modulation of Deq(t) has an amplitude only ∼1/2 as large as that of Dax(t). As a result, the total dipole moment and hence the IMF strength exhibit a broad minimum centered roughly on the polarity reversal Dax(t) = 0; conversely, they show a broad maximum around sunspot minimum, when Dax(t) is largest. The IMF variation in this "weak bipole" case is thus phase-shifted with respect to the "strong bipole" case of Figure 3.
Figure 4. Evolution of the solar magnetic field for the "weak bipole" case, where 100 bipoles each having flux Φ0 = 1 × 1022 Mx are deposited per 11 yr cycle.
Download figure:
Standard image High-resolution imageAs an explanation for the phase shift seen in the 10Be concentration, we therefore suggest that the active regions that emerged during the Maunder Minimum were systematically weaker than in the present day, leading to a reduction in the amplitude of Deq(t) relative to that of Dax(t). Such a decrease in the flux contained within each large bipole would also be consistent with the dearth of observed sunspots, given that a minimum field strength on the order of 1500 G is required for sunspot formation (see, e.g., Penn & Livingston 2006).
5. THE FIVE YEAR PERIODICITY
We now discuss the origin of the 5 yr periodicity detected in the 10Be record during cycles −4, −3, −2, 6, 8, 15, 16, and 17, whose peak sunspot numbers ranged from ∼46 (cycle 6) to ∼138 (cycle 8). Here, the IMF strength apparently decreased to low values at sunspot minimum as well as near sunspot maximum, so that the amplitude of Dax(t) cannot have exceeded that of Deq(t). If the equatorial dipole has comparable or greater amplitude, the shape of the IMF variation is determined largely by fluctuations in Deq(t), which in turn reflect fluctuations in the emergence rate and longitudinal locations of large active regions and activity complexes. As suggested by the simulations plotted in Figure 5(c) of Wang & Sheeley (2003a) and Figure 8 of Wang & Sheeley (2003b), the deposition of a small number of very strong bipoles at random longitudes, combined with a reduction in the poleward flow speed, often gives rise to two main peaks in Deq(t) during a given cycle. Here, the lower flow speeds lead to longer decay timescales for the equatorial dipole (which is annihilated by rotational shearing and diffusion as the flow transports the active region fields to midlatitudes), resulting in fewer but broader peaks. An additional factor that contributes to a double-peaked IMF variation is the polarity reversal of the axial dipole component, which may separate Dtot(t) into two distinct parts, even if Deq(t) shows multiple peaks.
To illustrate the basic effect of the polarity reversal, let the time variation of the axial and equatorial dipole components be represented by the idealized functions


where t is in years and 0 ⩽ t ⩽ 11. Fixing the amplitude a at 1 G, we calculate Dtot(t) = (D2ax + Deq)1/2 for b = 0.5, 1.0, and 1.5 G and plot the results in Figure 5. When the amplitude of the equatorial component is small compared to that of the axial component (b = 0.5a; Figure 5(a)), Dtot(t) shows a broad minimum centered on the point where Dax(t) vanishes, as in the "weak bipole" case of Figure 4. When the equatorial and axial dipoles have equal amplitudes (b = a; Figure 5(b)), Dtot(t) exhibits two broad, shallow dips, centered respectively on the cycle minimum and the polarity reversal. Finally, when the equatorial dipole dominates (b = 1.5a; Figure 5(c)), Dtot(t) has a single pronounced minimum located around sunspot minimum, and a broad maximum that dips slightly around the polarity reversal.
Figure 5. Effect of combining an axial dipole of the form Dax(t) = acos [2π(t/22)] with an equatorial dipole of the form Deq(t) = b{0.5tanh [(t − 1)/0.5] − 0.5tanh (t − 9)}, where t is in years. (a) a = 1 G, b = 0.5 G. (b) a = 1 G, b = 1 G. (c) a = 1 G, b = 1.5 G. When the axial and equatorial components have equal amplitudes, the total dipole strength Dtot(t) = (D2ax + D2eq)1/2 shows two minima, centered respectively on the cycle minimum and the polarity reversal. In reality, Deq(t) will undergo random fluctuations that depend on the strengths and longitudinal positions of large active regions.
Download figure:
Standard image High-resolution imageIn reality, of course, Deq(t) is not constant through the middle years of the cycle, but fluctuates on timescales of ∼1–3 yr. An example of the combined effect of the polarity reversal of the axial dipole and the random fluctuations of the equatorial dipole may be seen in the simulation of Figure 3. Here, during the first of the four 11 yr cycles, Deq(t) exhibits three main peaks. Because the middle peak is centered on the polarity reversal (Figure 3(b)), it is barely visible in the radial IMF strength, which shows only two main peaks with a broad dip occurring in between (Figure 3(c)). In contrast, during the third 11 yr cycle of the simulation, Deq(t) again shows three main peaks, but the valley between the second and third peaks coincides with the polarity reversal, so that all three peaks are preserved in the total dipole and radial IMF strength.
6. SUMMARY AND DISCUSSION
We now recapitulate the main points of this paper.
-
1.Because of their miniscule dipole strengths (∼10−6 G) and random axial orientations, ephemeral regions do not provide any measurable contribution to the Sun's open flux, despite their large numbers. Thus they cannot have been the source of the IMF during the Maunder Minimum. This conclusion is supported by the fact that the emergence rate of ephemeral regions shows very little variation over a solar cycle, whereas an 11 yr modulation was present in the 10Be record through the Maunder Minimum.
-
2.Present-day solar wind measurements, when combined with mass and energy conservation and extrapolations of the observed photospheric field, show that the mass and energy flux densities at the coronal base increase almost linearly with the footpoint field strength, but that the wind speed itself is uncorrelated with the latter. Thus, if the radial IMF strength was ∼4–7 times weaker during the Maunder Minimum than in recent times (as suggested by model reconstructions), the solar wind mass flux and proton density would also have been ∼4–7 times smaller, but the wind speed would not have changed significantly.
-
3.The phase shift observed in the 10Be time series during the Maunder Minimum, which indicates that the IMF strength peaked near the minimum rather than the maximum phase of the 11 yr cycle, can be explained by a decrease in the amplitude of the Sun's equatorial dipole component relative to that of its axial dipole component. Such a decrease could occur if the active regions emerging during the Maunder Minimum were systematically weaker than those in the present day.
-
4.The 5 yr periodicities seen in the 10Be record during several low and medium-high amplitude cycles can be accounted for by random fluctuations in the Sun's equatorial dipole component. Conditions that favor the formation of two main peaks include flux emergence dominated by small numbers of very strong active regions, and low meridional flow speeds (leading to longer decay timescales). In addition, the polarity reversal of the axial dipole component sometimes (when it occurs in phase with the fluctuations of the equatorial dipole) produces a dip in the total dipole and IMF strength near sunspot maximum.
Our basic conclusion is that the evolution of the Sun's large-scale field during very low amplitude cycles does not differ fundamentally from that during higher amplitude cycles, except that the much smaller amount of flux emerging in active regions leads to a substantially weaker IMF and more tenuous solar wind. An important question yet to be resolved is the nature of the active regions during grand minima. In general, it is the strongest bipoles of a given cycle (those having the most flux and the widest pole separations) that determine the Sun's dipole moment and its evolution. To account for the phase shift in the IMF variation during the Maunder Minimum, we have suggested that the largest bipoles at that time were systematically weaker than their counterparts in the present era. If this was indeed the case, it may be that only a small fraction of such bipoles contained detectable sunspots, and therefore that the amount of emerging flux is considerably underestimated if it is taken to be proportional to the recorded sunspot numbers. This question might be addressed using contemporary observations by measuring the magnetic flux contained in newly emerged bipoles and determining the threshold value below which sunspots are no longer present, as a function of the overall level of solar activity.
This work was instigated by discussions at the International Space Science Institute team workshop on "Long-Term Reconstruction of Solar and Solar Wind Parameters" (2012 May), and was funded by NASA and the Office of Naval Research.